The maximal exercise oxygen uptake (VO2 max) response physiologically reflects the clinical status of patients with low-output left heart failure (HF). The failure of VO2 max to rise above 12 ml/kg/min is a hallmark of deteriorated clinical status, impaired oxidative metabolic capacity and indicates advanced medical therapy is required to prolong life.1,2 The continuous-flow left ventricular assist device (cf-LVAD) is a progressive cutting-edge therapy increasingly recommended to end-stage patients for the management of signs and symptoms of HF, including impaired VO2 max.1–7
Contemporary evidence also suggests that while cf-LVAD support extends life, intentional device optimisation methods, including pump-speed modification during exercise, yield ambiguous and often confusing interpretations of VO2.1,4–7,8–13 Without the assurance that VO2 will increase post-implantation, it remains uncertain which key elements, beyond enhanced forward flow, contribute to VO2 and oxidative metabolic capacity in cf-LVAD recipients.8–16 What has yet to be examined in depth is how the exercise physiology of VO2 and physical O2 transport between the central and peripheral systems are impacted by artificial forward flow.
This article examines the physical elements of exercise physiology that should be considered when evaluating the aerobic exercise capacity of patients in advanced HF who are dependent on axial cf-LVAD support. As adjustable forward flow is a key feature of cf-LVAD therapy, particular emphasis is placed on the impact that pump speed has on the physical and physiological features of whole-body O2 transport and exercise VO2. Although studies have tested the effect of centrifugal cf-LVAD support on VO2, this research is not reviewed herein as the literature is too complex for a combined discussion involving axial flow devices. The unique mechanical and physiological properties of centrifugal versus axial flow require special consideration when interpreting the impact pump-speed optimisation methods can have on VO2.17–19
Mass and Local Oxygen Transport
The prominent and integrative failure of heart rate, stroke volume, preload and afterload, arterial pressure and systemic vascular resistance prevent cardiac output (Q) and physical O2 transport from intrinsically and reliably accommodating sudden changes in the oxidative metabolic demand of skeletal muscles in cf-LVAD candidates. These physiological interactions are highly complex. Even after the introduction of mechanical circulatory support, patients are not immune from physical O2 transport limitations that will impact VO2.3,8–16,20–23
Despite similar patient phenotypes, physical haemodynamic constraints and modest margins for physiological adaptation due to advanced whole-body disease, cf-LVAD therapy considerably reduces the debilitating physical consequences of HF in select recipients.3,8–11,13–16,20–23 However, it is not only physical contributions from classical determinants of central haemodynamics that are involved in such changes. Peripheral haemodynamics and the local and micro-level O2 transport environment play an underappreciated role in affecting aerobic exercise capacity, as demonstrated by the signs and symptoms of patients with refractory HF, including the worsening maximal VO2 seen in some patients post-implant.3,16,24–30
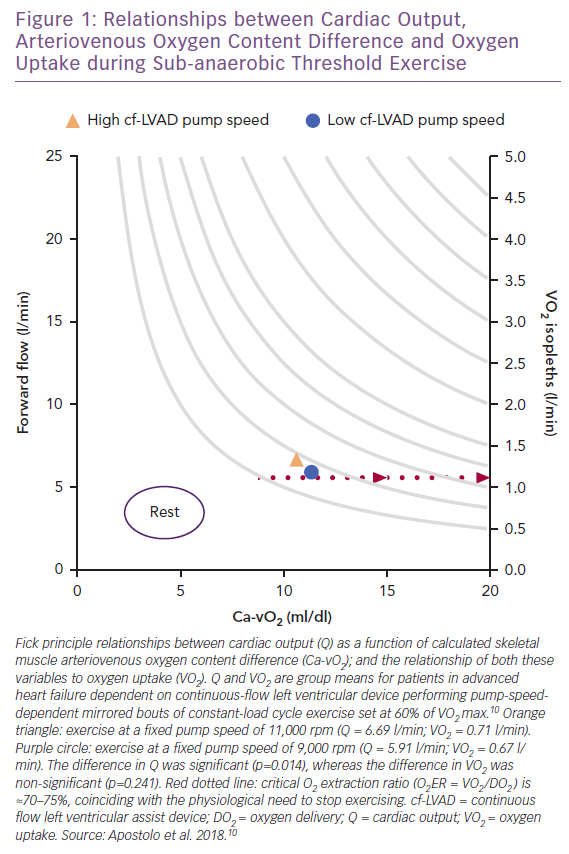
Haemodynamics: Convective and Conductive (Diffusive) Oxygen Transport
Despite obvious links between the rise in oxidative metabolic demand of skeletal muscle and increase in Q (with subcomponents) during exercise, Q only represents a portion of the contribution of physical O2 transport to VO2. Convective O2 delivery (DO2) to skeletal muscle, microcirculation and hundreds of square meters of capillary surface area required for O2 diffusion (DMO2) – the other major component of O2 transport – is also greatly dependent on physical chemistry. The O2-carrying capacity of blood and arterial O2 content (CaO2), both of which are signalled by oxyhaemoglobin (O2Hb) dissociation curve dynamics, play pivotal roles in facilitating the physical elements of VO2.24–28,31–33 Indeed, the confluence of cardiovascular system contributions to O2 transport is estimated to account for at least 70% of aerobic exercise capacity.31 Thus, it is not by unilaterally increasing total Q that tissue-specific peripheral haemodynamics, DO2 and DMO2 successfully meet the rapid and maximal O2 needs of mitochondria and related processes.24,26,28,34–36 For most adults, the ‘bulk O2 flow’ response (i.e. Q) to physical exertion does not typically limit exercise until high-metabolic-demand phases are achieved (i.e. the isocapnic buffering period is reached or exceeded); nor should it be expected that DO2 itself limits aerobic exercise.24,25,33,34,37–39
Current data suggest that while the mechanised central circulation undoubtedly works to recover part of the impaired native forward flow, this action alone cannot be assumed to translate to and coincide with equivalent and interactive improvements in DO2, DMO2 and/or VO2.3,8–11,14,15,20–23 Examining the nature of convective and conductive O2 transport relationships will be crucial in better understanding VO2, how to optimise pump speeds and where to focus when evaluating limitations in exercise.24–29
Optimising Fixed Pump Speed
Submaximal Exercise
Vignati et al. and, more recently, Apostolo et al. from the same group have comprehensively studied the impact of cf-LVAD speed (maximum rpm 12,000; flow rate 7 l/min) on upright aerobic exercise performance.9,10 Fick principle calculations were used to study how total Q (via inert gas rebreathing) changed as a function of adjustments in arteriovenous O2 content difference (Ca-vO2). For 15 patients who performed two mirrored bouts of sub-anaerobic threshold constant-load cycle exercise (mean 35 W), decreased pump speed from 11,000 rpm to 9,000 rpm translated into down-and-right-shifted coupling between Ca-vO2 and VO2 without affecting heart rate (Figure 1).10 This also means that Fick principle-derived VO2 isopleths (e.g. 1.25 l/min in Figure 1), can be taken to suggest increased Q from low-to-high pump speed is perhaps unnecessary given that VO2 was not impacted by pump speed while remaining proportionate to Ca-vO2.10
The varying sensitivities of Q, Ca-vO2 and VO2 to pump speed dynamics further suggests that within the rpm ranges studied, not only does the rate of forward O2 flow differential have a negligible impact on sub-anaerobic threshold VO2, but it is also possible that harm can be caused when pump speed is increased beyond that required to meet oxidative metabolic demand and overcome afterload.40–42 Excessive central-to-peripheral haemodynamic redistribution can overload hydrostatic pressure in the arterial and venous ends of skeletal muscle capillaries, resulting in exaggerated outward fluid flux, reduced inward fluid flux and a physical barrier to O2 transport.43–45
Based on what is already known about Starling forces and the determinants of O2 diffusion at the alveolar–capillary membrane interface, poor filtration and uncontrolled capillary hydrostatic pressure alongside imbalanced preload sensitivity to changes in afterload could be expected to result in or exacerbate the accumulation of peripheral interstitial fluid.46–53 Here, even a relatively small rise in capillary hydrostatic pressure, particularly at the venous end, would disrupt interstitial fluid balance.47,52
Measured changes in arterial pressure during exercise are also unlikely to be sensitive enough in cf-LVAD recipients to detect incremental changes in peripheral interstitial fluid accumulation that would limit DMO2. While this hypothesis has yet be tested in cf-LVAD recipients, the implication of rapid interstitial fluid accumulation is that the resulting physical barrier reduces DMO2 and leads to inadequate O2 transport to the mitochondria for oxidative phosphorylation.46–50 Thus, in the acute setting, it can be hypothesised that limitations to aerobic exercise in cf-LVAD recipients can be physical at the peripheral level and partly reversible by optimisation of pump speed relative to work-rate.
When No Increase in Submaximal VO2 is Beneficial
Based on available data, it is unclear whether pump speed-mediated increases in sub-anaerobic threshold VO2 for a fixed work-rate are beneficial.10 When patients performed mild-to-moderate intensity exercise they did not demonstrate pump speed reliance, and hence O2 flow-dependent reliance, on physical O2 transport (e.g. Q) for VO2.10 This result is consistent with the findings for healthy adults.24,25 During sub-anaerobic threshold exercise, cellular and biochemical pathways involving the mitochondria and oxidative phosphorylation primarily drive VO2.24,25 Thus, since no appreciable differences in sub-anaerobic threshold VO2 occurred with the change from low to high pump speed,10 it is possible that despite the potential to deliver more O2 on a rapid basis via cf-LVAD support, the metabolic necessity for O2 can still in part be regulated, even in severely advanced HF.
Numerous exercise studies across disciplines also report that the amount of excess VO2 for a given work-rate is key for assessing aerobic exercise capacity.54–60 A high VO2:W ratio (i.e. >10 ml/min VO2 per W) signals an inappropriate ‘gain’ in VO2 closely linked to metabolic inefficiency and supra-reliance on substrate-level phosphorylation for energy regeneration.54–60 This means that although the sub-anaerobic threshold VO2 is not significantly impacted by pump speed, the ‘gain’ in VO2 (calculated herein) increases at high versus low pump speed (20.3 versus 19.0 ml/min/W, respectively), suggesting exercise metabolic efficiency is slightly better when performed at lower pump speed.10 Thus, while the ‘gain’ in VO2 for both pump settings was greater than expected for comparable healthy adults,54–60 the interpretability of VO2 within any phase of exercise can be improved when evaluated relative to power.
Maximal Exercise
When maximal exercise data are plotted with submaximal data, see Figure 2, it appears that the insensitivity of VO2 to physical O2 transport during mild-to-moderate intensity exercise does not persist to maximal stress.9,10 At maximal exercise there was a better balance between Q and VO2, which coincided with a high pump speed (11,000 rpm). This observation is consistent with the increased VO2 max linked to ‘responsive’ residual left ventricular function (indicated by a resting left ventricular ejection fraction ≥40%) at high pump speed (9,000 rpm) in the HeartMate II treadmill studies.12
However, the impact of pump speed on coupling between VO2 and Ca-vO2 did not differ at maximal versus sub-anaerobic threshold exercise.9,10 The exact reasons for why VO2 demonstrated higher sensitivity to Q as opposed to Ca-vO2 at maximal exercise (ΔVO2 52 ml/min; p=0.01) cannot be independently explained by pump speed. The same pump speed settings were used for both exercise intensities.9 Maximal heart rate also did not differ between pump speeds, consistent with the heart rate responses reported in HeartMate II studies.8,12 Thus, peripheral haemodynamics, oxidative metabolic demand and skeletal muscle O2 transport mechanisms should not be overlooked as key interactive features linking pump speed and forward flow to changes in VO2 at maximal exercise.
Without reviewing additional data it can only be hypothesised that as oxidative metabolic demand of skeletal muscle rises towards maximal exertion in cf-LVAD recipients – coinciding with increased skeletal muscle pump activity, speed and frequency of venous return and exercise power – so does skeletal muscle recruitment.9,10,12,61 Therefore, increased total metabolic demand plus greater muscle recruitment should demonstrate a closer association with high rather than low pump speed assuming there is also negligible residual left ventricular function. As such, understanding the relationship between metabolically active skeletal muscle relative to total Q reveals the potential impact the microcirculation can have on DO2, DMO2, Ca-vO2, VO2 and exercise capacity.29,62 The evaluation of cf-LVAD recipients’ aerobic exercise capacity should include an integrated assessment highlighting the extent of multilevel (un)coupling across pump speed, forward flow, VO2, exercise power, skeletal muscle availability and recruitment.
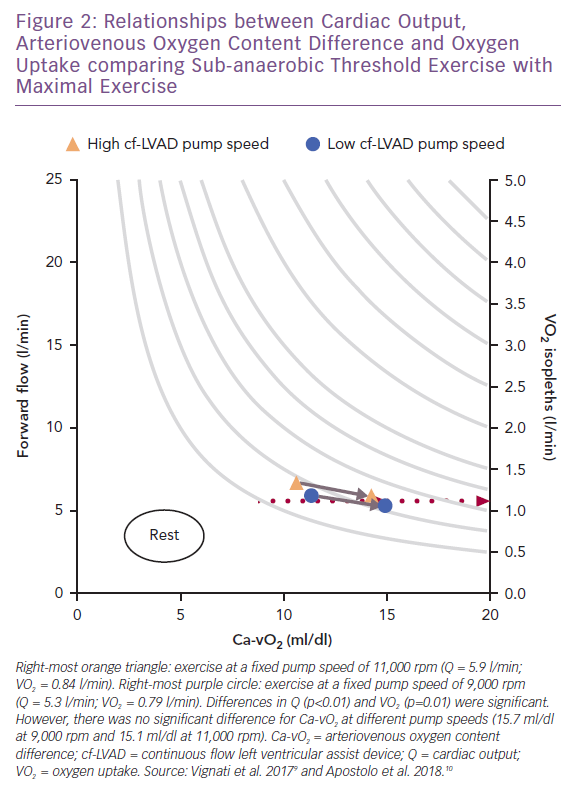
Skeletal Muscle Recruitment and Maximal Exercise
The ability to recruit skeletal muscle during aerobic exercise involves the collateral recruitment and perfusion of capillaries. In comparison to rest, the larger total capillary surface area available during exercise allows skeletal muscle to accommodate increased Q, and with this a greater potential for maximising physical O2 transport.
Viewing the collective differences between maximal Q, VO2 and Ca-vO2 as exercise capacity and clinical status worsen from Weber class A to D (Figure 3), a widening of Ca-vO2 reflects not only the peripheral response to oxidative metabolic demand, but also information concerning the inseparable links between Q and active skeletal muscle that allow DO2 and DMO2 to serve as physical determinants of VO2.63,64 Thus, when the recipient (i.e. skeletal muscle) of enhanced forward flow is appropriately responsive (e.g. capillaries are recruited), cf-LVAD support can be highly effective for improving aerobic exercise capacity.
Unfortunately, for most end-stage patients, the capacity for proper Ca-vO2 expansion relative to increasing oxidative metabolic demand is compromised by skeletal muscle disease(s). This clinical phenotype is not unique to advanced stage HF and can include cachexia, capillary rarefaction, low capillary surface area reserve, small type I-to-II muscle fibre ratios and, in most cases, a composite of these.64–70 Therefore, with the high likelihood that skeletal muscle pathophysiology progressively worsens in the lead-up to cf-LVAD support, ‘peripheral’ components of HF reinforce the view that whole-body physical O2 transport and aerobic exercise capacity can only be partially restored with artificial forward flow. Indeed, beyond cf-LVAD support alone, post-implantation participation in cardiac rehabilitation – including regular aerobic and strength training exercise – can lead to significant improvements in VO2 max.3,16,22,23
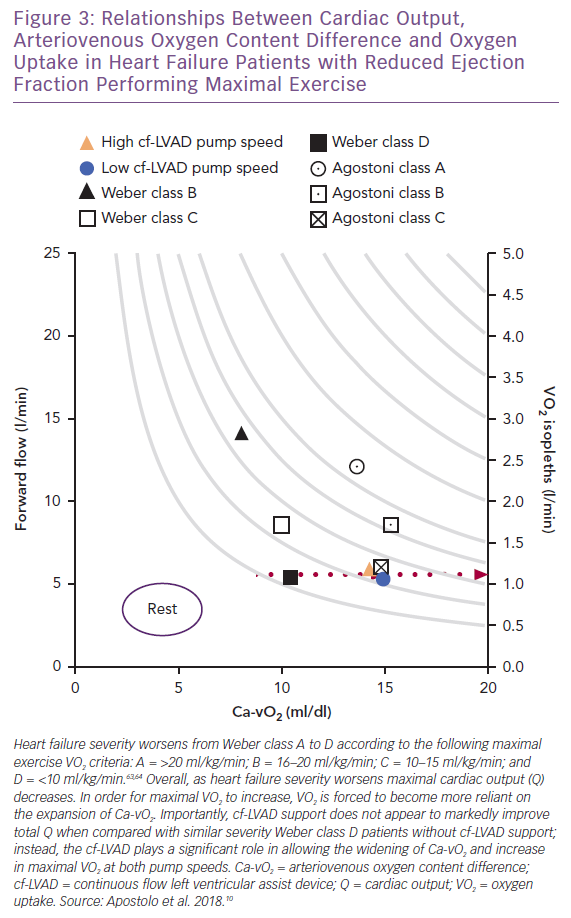
Is an Increase in Maximal VO2 an Improvement?
The acute ‘benefit’ of high pump speed on VO2 max may be outweighed by the negative impact long-term excessive rapid blood flow can have on shortened O2 microcirculatory transit times, imbalanced Starling forces and dysregulated hydrostatic and osmotic pressure gradients. Similar to what has been reported for the cardiopulmonary circulation, it can be hypothesised that for optimal coupling between Q, DO2 and DMO2 to occur in skeletal muscle, blood transit time needs to allow O2 to diffuse from the O2-rich microcirculation, across the membrane barrier, to the O2-poor interstitial fluid and finally the mitochondria.27,29,51,71
In the absence of an adequate capillary-to-interstitial partial pressure of oxygen (PO2) gradient, O2 microcirculatory transit time and capillary surface area, it would be nearly impossible for DMO2, O2 transport to mitochondria and oxidative phosphorylation to occur. This is because high hydrostatic pressure of the interstitial space, precipitated by capillary flooding secondary to excessive pump speed, would prevent DMO2. Moreover, if pump speed is too rapid to allow arterial reservoirs to fill, the impact the skeletal muscle pump has on shuttling venous return will be marginalised.72 This will result in progressively worsening preload to overcome high left-heart afterload.53 Thus, while transient low-to-high shifts in pump speed may temporarily increase VO2 max, whether this symbolises physiological adaptation, what is considered a sustainable ‘improvement’ in aerobic exercise capacity and the associated clinical benefit should be interpreted with caution.8–10,12
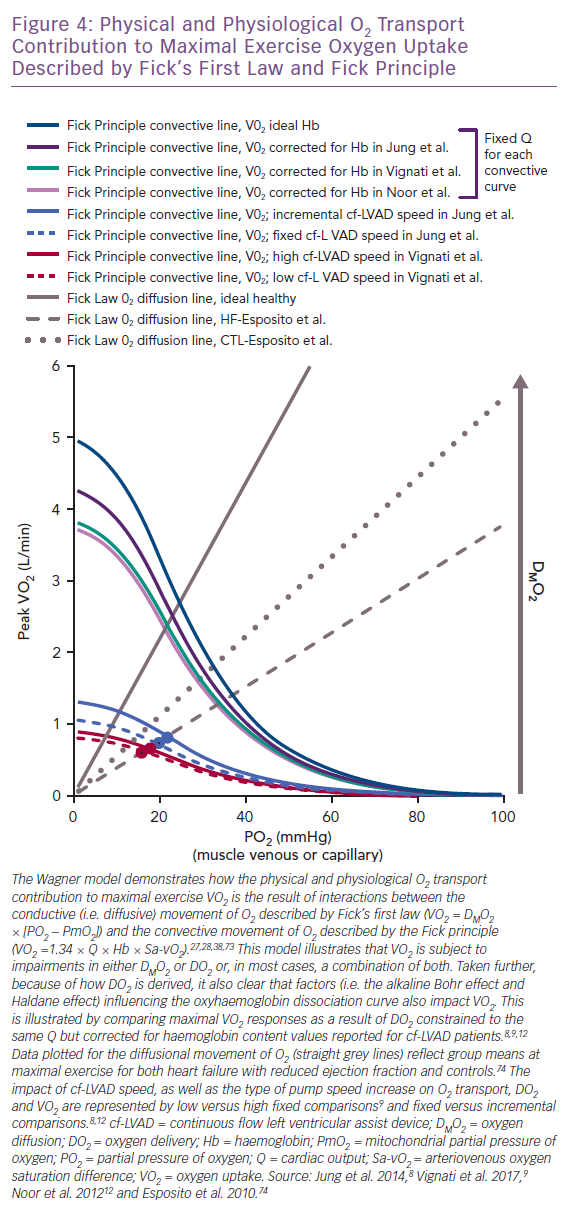
Linking Convective and Diffusive Oxygen Transport
DMO2 at skeletal muscle during exercise, as it relates to VO2, can be closely estimated using Fick’s first law.27,28,38,73 The equation
VO2 = DMO2 × (PO2 − PmO2)
suggests the intra- to extra-capillary flux of O2 is directly proportional to its conductance coefficient constant and a known PO2 gradient between the capillaries and mitochondria (PmO2).27,28,38,73 The foundational Wagner model for physical O2 transport was the first to illustrate how DMO2 integrates with elements underlying the Fick principle (VO2 = 1.34 × Q × Hb × arteriovenous oxygen saturation difference) and the O2Hb dissociation curve to jointly compose VO2.27,28,38,73 The interdependence of such relationships, where VO2 is derived using both the Fick principle and Fick’s first law, are plotted together in Figure 4 as a function of the expected change in skeletal muscle PO2 accompanying maximal exercise (capillary [PcO2], PvO2 or mixed venous [PmvO2]) .27,28,38,73 The DMO2 and DO2 lines intersect closely, representing the total physical contribution of O2 transport to aerobic exercise capacity, regardless of patient type.12,27,28,38,73
Indeed, if maximal exercise DMO2 (mean 12.6 ml/min/mmHg) is set at levels reported for HF without cf-LVAD74, the point where DMO2 (grey hashed line) and DO2 (blue and red curved lines) intersect for cf-LVAD (Figure 4) illustrates failing DMO2 and DO2 together but not separately physically limit VO2.8–10 Given the Fick principle, the direct impact that O2Hb association and dissociation capacity have on DO2 and subsequently DMO2 is also apparent. This physical chemistry factor is capable of predetermining the VO2 ceiling, irrespective of pump speed and total Q.12,27,28,75,76 To illustrate this point, the DO2 curves (light purple, teal and dark purple) corrected for haemoglobin (mean 11.2 g/dl, 11.5 g/dl and 12.9 g/dl) demonstrate a clear downward shift from the ideal DO2 curve (dark teal) reflecting normal haemoglobin (15.0 g/dl; Figure 4).8,9,12 The ensuing differences in VO2 reflect the robust influence of the O2Hb dissociation curve independent of haemodynamic differences, since Q was set at the same fixed constant for DO2 curves.
If DMO2 increases towards levels reflective of healthy controls (mean 18.5 ml/min/mmHg; grey dotted line in Figure 4), it becomes apparent that improving DMO2 by increasing capillary unit and surface area recruitment could compensate for low DO2 and facilitate a rise in VO2.74 However, the promise of this compensatory mechanism means it is unlikely that further increases in DMO2 are plausible for cf-LVAD recipients if the level for HF reported in Esposito et al. even slightly overestimates what could be expected for more severe HF.74 This is because the critical exercise termination thresholds for the O2 extraction ratio (O2ER = VO2/DO2 ≈ 70–75%) and PO2 for capillary and femoral venous blood (<25 mmHg) align vertically at the intersection of the HF DMO2 line and DO2 curves.71,77 Thus, all of this collectively suggests that true maximal VO2 was achieved by cf-LVAD recipients in reviewed studies and no further increase in DMO2 could be expected to safely occur.
Does Incremental Pump Speed Improve Exercise Capacity?
In contrast to optimising the cf-LVAD for exercise using a fixed pump speed,9,12 a number of studies have tested whether modifying pump speed (HeartMate II maximal rpm = 15,000; flow = 10 l/min) between fixed and incremental settings impacts maximal VO2 and Q.8,11,13 In Figure 5, separate upright cycle maximal exercise data for incremental pump speeds of +200 rpm/min and +400 rpm/2 min (maximal mean for both = 10,843 rpm) suggest similar VO2, cf-LVAD flow, Ca-vO2, heart rate and W responses.8,13 Conversely, the study by Fresiello et al. reported that pump speed type had no effect on VO2, whereas Jung et al. identified an increased VO2 response associated with incremental pump speed, consistent with observations made by Apostolo et al.8,10,13
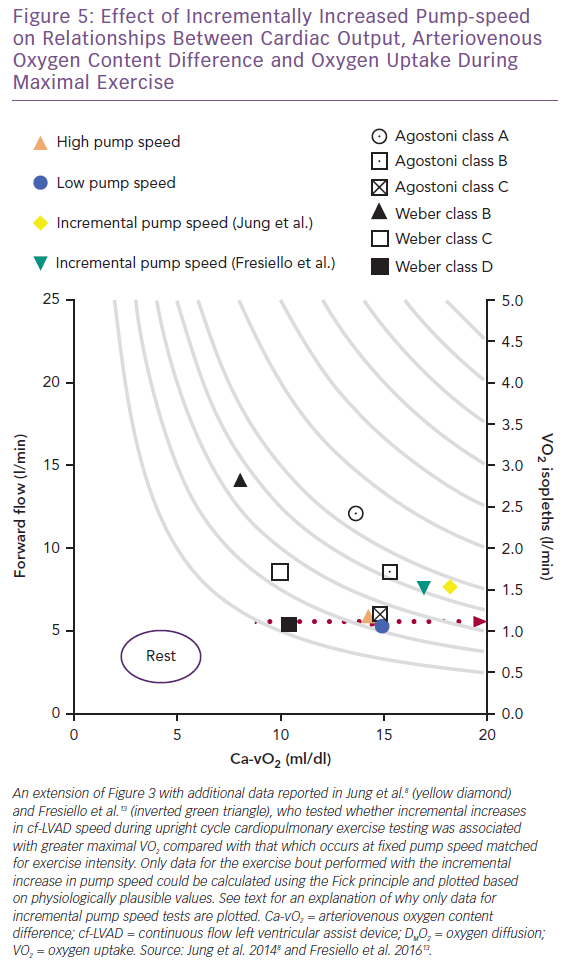
Interestingly, Fick principle data for VO2 (1.22 and 1.27 l/min) and cf-LVAD flow (6.0 and 6.2 l/min) could not be plotted for fixed tests at 9,371 and 9,357 rpm as values calculated for Ca-vO2 were atypically high (20.3 and 20.5 ml/dl, respectively).8,13 Even when considering that the effects of the O2Hb dissociation curve and variability in reported data are not accounted for by calculating Ca-vO2 using means, it is physiologically unlikely that Ca-vO2 was supra-responsive and mixed venous O2 saturation drastically reduced during fixed versus incremental studies. Based on available data and the Fick principle, it can only be hypothesised that subtle contributions from native Q during fixed tests makes up for lower cf-LVAD flow while reducing Ca-vO2 to more plausible levels. Native Q may make different contributions to gross flow depending on pump speed type and right and left heart interactions involving unique cf-LVAD sensitivity to preload and afterload, as briefly discussed below.
Right Heart Considerations when Interpreting cf-LVAD Contributions to Aerobic Exercise Capacity
In lieu of a strong body of evidence to support the current understanding of right heart function during exercise testing in cf-LVAD recipients, in silico studies suggest the cf-LVAD exhibits a considerably lower preload sensitivity than the intact human heart.53,78 On the other hand, simulations demonstrate that, within a given patient, left heart preload sensitivity lessens as afterload increases whereas left heart afterload sensitivity is nominally impacted by changes in preload.53 All of this means that, while right ventricular function and preload have a clear impact on cf-LVAD function, proposed associations between preload and afterload suggest the strongest interactions occur when the afterload is highest.6,53,79 However, afterload, even in cases of advanced stage HF, does not necessarily peak at maximal exercise or relate to whether native left ventricular function is able to contribute to ‘normal’ aortic valve function.8,12,80–82 Classical resting evaluations of right heart function may not fairly indicate the heart’s ability to perform under conditions where the active skeletal muscle pump and drop in afterload have favourable influences on haemodynamics.
On a real-time basis, non-physiological elements confound what is understood about the influence right heart function has on left heart forward flow and VO2. For example, low preload sensitivity during exercise has implications for cf-LVAD haemodynamics because the presence of high afterload must be offset by adequate venous circulation and right ventricular function in order to decrease the chance of ‘suckdown’.53,78 This also means factors, such as gravity and body position, which directly affect the venous circulation must be taken into account when evaluating exercise and right heart function in cf-LVAD recipients.40,83–86 Collective effects associated with venous return, right ventricular function, skeletal muscle pump activity and left heart afterload are likely to have less of an impact on how cf-LVAD support integrates with native Q and gross physical O2 transport during supine exercise in comparison to the upright position.40,83–86 Caution should be taken when translating (semi)supine studies to the upright position, particularly when evaluating the combined effects of right ventricular function, native Q and cf-LVAD support on the various subcomponents of physical O2 transport and aerobic exercise capacity.
Conclusion
The physiological concepts proposed in this review suggest that for cf-LVAD pump speed transitions to be effective and safely impact VO2 and aerobic exercise capacity, the rapidity of the pump must not be set so the rate of forward flow grossly exceeds skeletal muscle oxidative metabolic demand, microcirculatory net filtration capacity and exercise work-rate. The unique role skeletal muscle plays in accommodating and facilitating physical O2 transport and performing oxidative metabolism highlights the therapeutic role that cardiac rehabilitation and aerobic exercise training can have on maximising the benefit of cf-LVAD support. Clinical and mechanistic studies are needed to explore and test the validity of the proposed links between pump speed, forward flow, whole-body physical O2 transport, and the physical and physiological features of skeletal muscle when oxidative metabolic demand is expected to be highest, during maximal exercise.